Theoretical Background
Dye-Sensitized Solar Cells (DSCs) represent a third-generation photovoltaic technology that converts light into electricity through a unique approach, leveraging molecular dyes to capture photons instead of relying on traditional semiconductor materials. Originally developed by Michael Grätzel and Brian O’Regan in 1991, DSCs mimic photosynthesis in plants, using a dye to absorb light and initiate an electron flow. When exposed to light, the dye molecules inject electrons into a porous layer of titanium dioxide (TiO₂), which then conducts the electrons toward an electrode, creating an electric current. This simple, efficient mechanism makes DSCs particularly suited for environments where light intensity is low, such as indoor settings.
Indoor Photovoltaics for the Internet of Things (IoT)
IPV is a predictable, controllable and widely available energy source in indoor conditions. It can provide power densities between 10-5 and 10-3 W/cm2.[1] A suitable photovoltaic device under ambient lighting should perform efficiently under light intensities of around 200 (living room environment) and 2000 lux (office environment), corresponding to approximately 0.1-2 % of the standard 1-sun AM1.5 solar irradiation. Furthermore, most artificial indoor lighting sources emit in the visible part of the spectrum, between 400 and 700 nm.[2][3] (See Figure 1). Hence, organic dyes that collect photons in this part of the spectrum are required for efficient IPV.
Figure 1: Spectra of typical artificial lighting sources (right) and solar spectrum at standard conditions (left). (Solar Energy Materials and Solar Cells
Volume 90, Issue 14, 6, 2006, 2141-2149)
As the EU has recently banned fluorescence light, LED will be the most employed artificial light source in the mid and the long-term. It can be demonstrated that the optimum band gap for a typical LED artificial light source is 2 eV and the maximum theoretical efficiency achievable under the assumptions of detailed balance (radiative recombination limit) is about 50- 60%.[4] The core functional requirement is to power household devices and enable IoT nodes to provide a sufficient and reliable electricity supply. In Table 1, details of some application examples are collected.
Device | Power consumption (W) | Required IPV area (200 lux)* | Required IPV area (1000 lux)* |
Small calculator | 3×10-6 | 0.08 cm2 | 0.016 cm2 |
Passive Wi-Fi | 10-4 | 2.5 cm2 | 0.5 cm2 |
CO detector/ Bluetooth | 10-3 | 25 cm2 | 5.0 cm2 |
LED | 10-1 | 0.25 m2 | 500 cm2 |
Smartphone/Wi-Fi emitter | 1 | 2.5 m2 | 0.50 m2 |
*For a 20% efficient photovoltaic solar cell, LED white lamp.
In Table 1, areas required to power several small electronic devices with a reference value of 20% efficiency are shown. Increasing the efficiency closer to the maximum theoretical efficiency (i.e.> 35-40%) would reduce the required areas by almost a half, enabling easier implementation and reducing materials needs.
Beyond energy efficiency, IPV must prioritize sustainability, minimize environmental impacts in terms of toxicity and global warming, and adhere to the ‘Do No Significant Harm’ principle. This principle applies to sourcing base materials, manufacturing, usage, and end-of-life decommissioning. Additionally, IPV devices need to be highly durable, and functioning well for several years to compete with technologies that require battery replacements. In essence, IPV is envisioned to power IoT devices perpetually, ensuring reliability and minimal waste generation.
Household and IoT devices require voltages of up to 5 V. One of the potential advantages of IPV is, due to the above-mentioned features of the artificial lighting sources (more concentrated in the visible part of the spectrum), that materials and molecules with larger band gaps are best suited. This makes it possible to reach relatively high voltages despite the low light intensity. In particular, the DSC concept is especially advantageous by itself in comparison with other technologies. To further improve the voltage at low illumination levels, and because of the logarithmic dependence of the open circuit voltage (Voc) with light intensity (I), it is very important to look at the ideality factor. This is a key quantity related to the recombination mechanism. For this reason, analysis and quantification of the recombination mechanism and the overall recombination loss in DSC is key to optimising voltage and the overall performance at low light intensities and under artificial lighting sources.
Unlike outdoor solar scenarios, low-power indoor photovoltaics (IPV) are less affected by series resistance losses, but shunt-resistance leaks become crucial. IPV requirements for stability are not as strict as those for high-throughput outdoor applications, yet resilience to humidity and other environmental factors is desirable. Indoor applications demand the ability to utilize variable and non-flat surfaces, making the flexibility and minimal dependence on the light’s angle of incidence key advantages of these IPV technologies.
Fundamentals of Dye-Sensitized Solar Cells
DSCs are composed of three main components:
- Light-Sensitive Dye: This dye is adsorbed onto the surface of TiO₂ nanoparticles, and upon light absorption, it releases electrons that enter the conduction band of the TiO₂.
- Semiconductor (TiO₂): The semiconductor, typically titanium dioxide, provides a high surface area for the dye to adhere and facilitates electron transport.
- Electrolyte: The electrolyte regenerates the dye molecules by providing them with electrons from the counter electrode, completing the circuit.
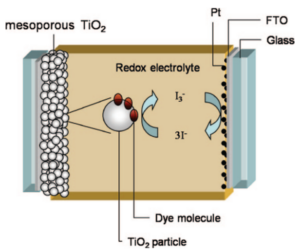
The typical basic configuration is as follows: At the heart of the device is the mesoporous oxide layer composed of a network of TiO2 nanoparticles that have been sintered together to establish electronic conduction. Typically, the filmthickness is ca. 10 µm and the nanoparticle size 10-30 nmin diameter. The porosity is 50-60%. The mesoporous layer is deposited on a transparent conducting oxide (TCO) on glass or plastic substrate. The most commonly used substrateis glass coated with fluorine-doped tin oxide (FTO). Attached to the surface of the nanocrystalline film is a monolayer of the charge-transfer dye. Photoexcitation of the latter results in the injection of an electron into the conduction band of the oxide, leaving the dye in its oxidized state. The dye is restored to its ground state by electron transfer from the electrolyte, usually an organic solvent containing the iodide/triiodide redox system. The regeneration of the sensitizer by iodide intercepts the recapture of the conduction band electron by the oxidized dye. The I3- ions formed by oxidation of I– diffuse a short distance (<50 µm) through the electrolyte to the cathode, which is coated with a thin layer of platinum catalyst, where the regenerative cycle is completed by electron transfer to reduce I3- to I–.
Indoor Applications of DSCs
DSCs have gained attention as a prime candidate for Indoor Photovoltaic (IPV) applications because they are particularly effective at capturing ambient light from artificial sources, such as LEDs and fluorescent lamps. Unlike conventional silicon-based solar cells performing best under direct sunlight, DSCs are uniquely efficient under low-light conditions. This makes them ideal for powering small devices indoors, such as sensors, IoT, and low-power electronics, without needing disposable batteries. The flexibility of DSCs in terms of design, colour, and transparency also opens up possibilities for integrating these cells into building interiors, furniture, and smart home systems.
Advantages of DSCs for Indoor Use
- High Efficiency under Low Light: DSCs excel under low illumination, such as indoor lighting, maintaining efficiency where other photovoltaic technologies, like silicon, struggle.
- Cost-Effective and Environmentally Friendly: DSCs use abundant and non-toxic materials, making them less costly and more sustainable to produce.
- Flexible Design Options: DSCs can be manufactured in various shapes, colors, and degrees of transparency, enabling aesthetic and functional integration into indoor spaces.
- Potential for Battery Replacement: By providing a stable, renewable power source for low-power devices, DSCs reduce the dependency on batteries, contributing to a decrease in electronic waste.